Implementation
13C NMR has a number of complications that are not encountered in proton NMR.
13C NMR is much less sensitive to carbon than
1H NMR is to hydrogen since the major isotope of carbon, the
12C isotope, has a
spin quantum number of zero and so is not magnetically active and therefore not detectable by NMR. Only the much less common
13C isotope, present naturally at 1.1% natural abundance, is magnetically active with a spin quantum number of 1/2 (like
1H) and therefore detectable by NMR. Therefore, only the few
13C nuclei present resonate in the magnetic field, although this can be overcome by isotopic enrichment of e.g.
protein samples. In addition, the
gyromagnetic ratio (6.728284 10
7 rad T
−1 s
−1) is only 1/4 that of
1H, further reducing the sensitivity. The overall
receptivity of
13C is about 4 orders of magnitude lower than
1H.
[1]

Another potential complication results from the presence of large one bond
J-coupling constants between carbon and hydrogen (typically from 100 to 250 Hz). In order to suppress these couplings, which would otherwise complicate the spectra and further reduce sensitivity, carbon NMR spectra are proton
decoupled to remove the signal splitting. Couplings between carbons can be ignored due to the low natural abundance of
13C. Hence in contrast to typical proton NMR spectra which show multiplets for each proton position, carbon NMR spectra show a single peak for each chemically non-equivalent carbon atom.
In further contrast to
1H NMR, the intensities of the signals are not normally proportional to the number of equivalent
13C atoms and are instead strongly dependent on the number of surrounding
spins (typically
1H). Spectra can be made more quantitative if necessary by allowing sufficient time for the nuclei to
relax between repeat scans.

High field magnets with internal bores capable of accepting larger sample tubes (typically 10 mm in diameter for
13C NMR versus 5 mm for
1H NMR), the use of relaxation reagents,
[2] for example Cr(acac)
3 (chromium (III) acetylacetonate, CAS number 21679-31-2), and appropriate pulse sequences have reduced the time needed to acquire quantitative spectra and have made quantitative carbon-13 NMR a commonly used technique in many industrial labs. Applications range from quantification of
drug purity to determination of the composition of high molecular weight synthetic
polymers.
13C
chemical shifts follow the same principles as those of
1H, although the typical range of chemical shifts is much larger than for
1H (by a factor of about 20). The chemical shift reference standard for
13C is the carbons in
tetramethylsilane (TMS), whose chemical shift is considered to be 0.0 ppm.
Typical chemical shifts in 13C-NMR
DEPT spectra
DEPT spectra of propyl benzoate
DEPT stands for
Distortionless
Enhancement by
Polarization
Transfer. It is a very useful method for determining the presence of primary, secondary and
tertiary carbon atoms. The DEPT experiment differentiates between CH, CH
2and CH
3 groups by variation of the selection angle parameter (the tip angle of the final
1H pulse):
- 135° angle gives all CH and CH3 in a phase opposite to CH2
- 90° angle gives only CH groups, the others being suppressed
- 45° angle gives all carbons with attached protons (regardless of number) in phase

Signals from quaternary carbons and other carbons with no attached protons are always absent (due to the lack of attached protons).
The polarization transfer from
1H to
13C has the secondary advantage of increasing the sensitivity over the normal
13C spectrum (which has a modest enhancement from the
NOE (Nuclear Overhauser Effect) due to the
1H decoupling).
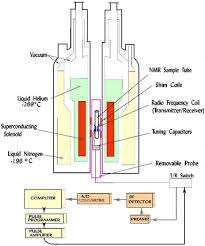
APT spectra
Another useful way of determining how many protons a carbon in a molecule is bonded to is to use an
Attached
Proton
Test, which distinguishes between carbon atoms with even or odd number of attached
hydrogens. A proper spin-echo sequence is able to distinguish between S, I
2S and I
1S, I
3S spin systems: the first will appear as positive peaks in the spectrum, while the latter as negative peaks (pointing downwards), while retaining relative simplicity in the spectrum since it is still broadband proton decoupled.
Even though this technique does not distinguish fully between CH
n groups, it is so easy and reliable that it is frequently employed as a first attempt to assign peaks in the spectrum and elucidate the structure.
[3]
- ^ R. M. Silverstein, G. C. Bassler and T. C. Morrill (1991). Spectrometric Identification of Organic Compounds. Wiley.
- ^ Caytan, Elsa; Remaud, Gerald S.; Tenailleau, Eve; Akoka, Serge, GS; Tenailleau, E; Akoka, S (2007). "Precise and accurate quantitative 13C NMR with reduced experimental time". Talanta 71 (3): 1016–1021. doi:10.1016/j.talanta.2006.05.075. PMID 19071407
- ^ Keeler, James (2010). Understanding NMR Spectroscopy (2nd ed.). John Wiley & Sons. p. 457. ISBN 978-0-470-74608-0.